The Information Mastery of Nature: Insights from Neuroscience
Written on
Chapter 1: Nature's Information Management System
Nature excels at managing information, which is the essence of life, with remarkable precision.

As the space race commenced in the 1960s, scientists encountered a perplexing question: how could they identify life on other planets, where biological evolution might differ significantly from Earth? James Lovelock, the originator of the Gaia hypothesis, suggested a key indicator: seek order. Each organism represents a brief emergence of structure from disorder, an intricate assembly that fiercely protects its organization until its demise. Maintaining this order relies heavily on sophisticated information processing, despite life emerging from chaotic materials such as erratic chemicals and convoluted polymers. This raises a question: should biological information be managed chaotically and inefficiently? Surprisingly, many biological computations exhibit such efficiency that they approach the theoretical limits of mathematical performance; this is our legacy.
Section 1.1: The Wonders of DNA
DNA is an astonishing medium for information storage, boasting a density surpassing that of any known format, from conventional hard drives to quantum systems. Incredibly, all the digital information on Earth could fit within a single dot of DNA weighing no more than eight paper clips. This extraordinary density is complemented by an equally impressive reading mechanism. For instance, during embryonic development, the embryo must guide the swift division, migration, and differentiation of its cells based on the DNA information. Cells differentiate based on their position within the embryo, necessitating precise control over gene expression across both spatial and temporal dimensions. Any minor inaccuracies could lead to the organism's death or malformation.
The pivotal question arises: how swiftly and effectively can spatial information be conveyed to ensure proper development? Alan Turing, a pioneer in modern computing, was intrigued by the possibility of reducing life to mathematical principles and explored this concept in the early 1950s. He proposed that the spatial organization of tissues during embryonic development could be modulated through the concentration of chemical signals known as morphogens. Turing's equations demonstrated that interactions between morphogens activating and inhibiting each other's gene expression could create stable waves of morphogen concentration, thereby influencing embryonic patterning. By manipulating just four variables—production and degradation rates, diffusion rate, and interaction strength—Turing could replicate biologically plausible, self-organized pattern formation.
The first video illustrates the challenge of crafting a nature wand, demonstrating how nature inspires creativity and problem-solving.
Section 1.2: Chemical Information Systems
Turing's hypothesis regarding a chemical information management system was validated decades later with the discovery of the bicoid morphogen in fruit flies. In a fly embryo, cells with a high concentration of bicoid develop into the head, while those with lower concentrations form the body. Notably, these cells differentiate with remarkable speed and accuracy. In 2007, a team led by Princeton biophysicist Thomas Gregor assessed the bicoid concentration gradient and diffusion rates in fruit fly embryos. They estimated that it would typically take around two hours for embryonic cells to gauge the morphogen concentration accurately enough to differentiate adjacent cells. However, this duration nearly encompasses the entire developmental timeline of the fruit fly, from fertilization to cellularization. The embryo was developing at a pace and precision that seemed almost miraculous.
Gregor hypothesized that cells might communicate using another signaling chemical, potentially a morphogen named hunchback. This would enable them to calculate a spatial average of bicoid concentrations, as opposed to relying solely on individual readings. By averaging these signals, the cells could mitigate the impact of noise and variations, achieving the necessary pattern accuracy in about three minutes instead of two hours.
The efficiency of this process is astounding. Physicist William Bialek utilized nucleus-by-nucleus assessments of morphogens in fruit fly embryos to determine how closely hunchback concentrations tracked changes in bicoid levels. His research revealed that the fidelity of this information transfer reached 90% of the theoretical maximum.
Chapter 2: Fractal Solutions in Biological Systems
As an embryo transforms into an adult, it faces new challenges in information processing. The organism must learn to sample and integrate both external and internal signals. For example, scent molecules may indicate a predator, prompting a rodent to escape, while the setting sun can trigger melatonin release, leading to sleep. Internal chemoreceptors detect elevated carbon dioxide levels, causing an athlete to breathe more rapidly. Here, information from tiny measurements gathered by diverse sensors must be synthesized to inform larger behavioral decisions communicated back to relevant body parts.
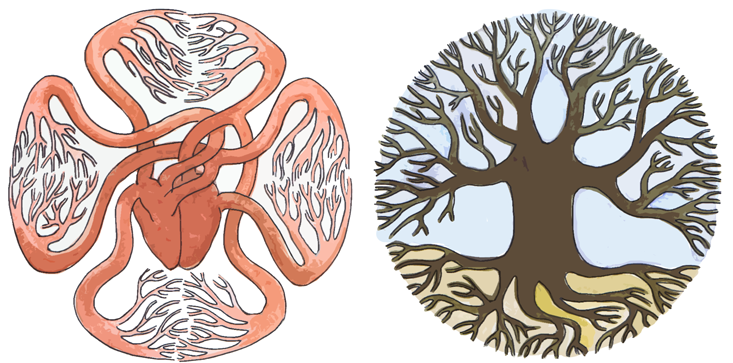
Nature, again, has crafted an optimal solution, evident in the anatomical structures of organisms. A fundamental observation in biology is that nearly every living being across ecosystems, spanning over 20 orders of magnitude from unicellular to multicellular organisms, exhibits features that scale with the organism's mass, such as heart rate, lifespan, aorta size, trunk size, and metabolic rate. This phenomenon is referred to as allometric scaling.
A common principle observed in biology is the power law relationship between these features and an organism's mass. For instance, metabolic rate is often proportional to the mass raised to a certain constant power. Intriguingly, this constant is frequently a multiple of one fourth. Physicist Geoffrey West posits that this scaling arises from the prevalence of fractal-like networks within organisms. Fractals are structures that maintain similar patterns across vastly different scales—an example being the branching structure of a leaf's vasculature.
Why does nature frequently employ fractal geometry? Mathematically, fractals possess a fractional dimension that exceeds the dimension of the space they occupy. For instance, a fractal drawn on a two-dimensional surface may have a dimension of 2.1. This characteristic allows nature to embed aspects of a fourth dimension within a three-dimensional realm.
To illustrate the utility of fractals, consider Pollack’s Rule in the semiconductor industry. It states that the speedup gained by adding processing elements to a chip is proportional to the count of added elements raised to the power of (1–1/D), where D represents the dimension of the arrangement. A three-dimensional configuration of 100 processors yields greater efficiency than a two-dimensional setup due to reduced signal latency. This principle similarly applies to biological systems, where a fractal arrangement enhances the speed of information processing.
The second video explores mastering plant identification, showcasing how interconnected ecosystems rely on information flow.
The Brain: The Apex of Information Processing
The brain epitomizes biological information processing and communication. Traditionally regarded as a noisy organ, neurons transmit information via electrical impulses known as spikes. When a neuron's voltage surpasses a specific threshold, it generates a spike, sending signals to downstream neurons. The fluctuations in the collective output of the brain's 100 billion neurons, as captured by an electroencephalogram (EEG), are often smaller than individual spikes and were historically considered merely reflective of brain states, rather than informative signals.
However, recent insights reveal that the so-called noise in EEG readings is, in fact, a vital signal that contributes significantly to neural computation. The variability in the brain's summed output influences the resting potential of neurons, affecting their likelihood of reaching the spike threshold. Slow oscillations in brain activity, observable during drowsiness or sleep, are coherent over extensive anatomical distances, much like how bass frequencies are perceived more clearly than treble.
This phenomenon facilitates communication between various brain regions. When a spike aligns with the peak of a slow amplitude fluctuation, it produces a more pronounced effect downstream than if it coincides with a trough. Neurons seem to synchronize their activity to create self-regulating global feedback, enhancing even subtle signals: no information is lost.
The notion of "waste" in the brain's context remains ambiguous. Theoretical neuroscientist Tony Bell contends that biological computation is characterized by information flowing through multiple layers of complexity, rising from microscopic to macroscopic levels and back down.
In this dynamic information flow, simpler structures, like neurons, interact with more complex systems, such as various brain areas, and vice versa. Compelling evidence suggests that even subcellular compartments within neurons engage in their computations. A recent study published in Nature in October 2013 demonstrated that dendritic activity shapes the information processed by the neuron.
This intricate information flow is not confined to the brain; it can also be observed in epigenetic processes. Bell asserts that this multidirectional information exchange defines life itself. Future advancements in artificial intelligence may not rely on silicon but could harness repurposed biological systems. To unlock this potential, a radically different scientific approach is needed to comprehend the higher-order, emergent capabilities of nature's self-organizing structures. While biomimicry holds immense promise, merely replicating physical designs may overlook the essence of nature: form is fluid, adapting to meet the demands of its environment. Understanding the foundational principles behind these ingenious biological solutions will ignite a true revolution.
Reference:
Smith, S., et al. Dendritic spikes enhance stimulus selectivity in cortical neurons in vivo. Nature 503, 115–120 (2013).
Kelly Clancy studied physics at MIT, then worked as an itinerant astronomer for several years before serving with the Peace Corps in Turkmenistan. She is currently a National Science Foundation fellow studying neuroscience at UC Berkeley.